A new view into prokaryotic cell biology from electron cryotomography
Electron cryotomography (ECT) enables intact cells to be visualized in 3D in an essentially native state to 'macromolecular' ( ∼ 4 nm) resolution, revealing the basic architectures of complete nanomachines and their arrangements in situ. Since its inception, ECT has advanced our understanding of many aspects of prokaryotic cell biology, from morphogenesis to subcellular compartmentalization and from metabolism to complex interspecies interactions. In this Review, we highlight how ECT has provided structural and mechanistic insights into the physiology of bacteria and archaea and discuss prospects for the future.
This is a preview of subscription content, access via your institution
Access options
Subscribe to this journal
Receive 12 print issues and online access
206,07 € per year
only 17,17 € per issue
Buy this article
- Purchase on SpringerLink
- Instant access to full article PDF
Prices may be subject to local taxes which are calculated during checkout
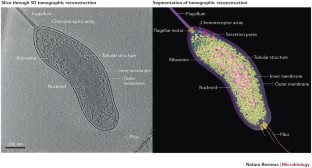

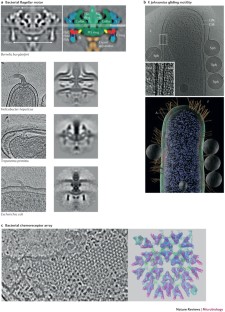
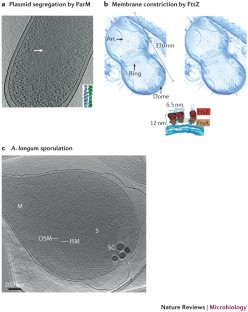
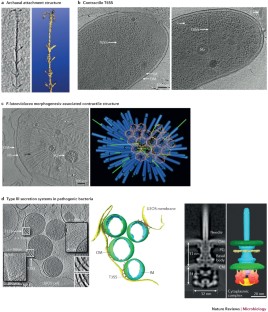
Similar content being viewed by others
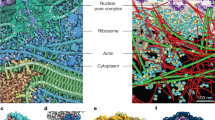
Bridging structural and cell biology with cryo-electron microscopy
Article 03 April 2024
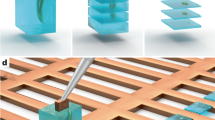
Serial Lift-Out: sampling the molecular anatomy of whole organisms
Article Open access 18 December 2023
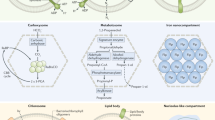
Formation and function of bacterial organelles
Article 24 July 2020
Change history
References
- Loewy, A. G. & Siekevitz, P. Cell Structure and Function (Brooks/Cole, 1991). Google Scholar
- Alberts, B. et al. Molecular Biology of the Cell (Garland Publishing, 1983). Google Scholar
- Goodsell, D. S. Escherichia coli. Biochem. Mol. Biol. Educ.37, 325–332 (2009). Google Scholar
- Alberts, B. The cell as a collection of protein machines: preparing the next generation of molecular biologists. Cell92, 291–294 (1998). ArticleCASPubMedGoogle Scholar
- Pilhofer, M., Ladinsky, M. S., McDowall, A. W. & Jensen, G. J. Bacterial TEM: new insights from cryo-microscopy. Methods Cell Biol.96, 21–45 (2010). ArticlePubMedGoogle Scholar
- Pilhofer, M. et al. Architecture and host interface of environmental chlamydiae revealed by electron cryotomography. Environ. Microbiol.16, 417–429 (2014). ArticleCASPubMedGoogle Scholar
- Dubochet, J. et al. Cryo-electron microscopy of vitrified specimens. Q. Rev. Biophys.21, 129–228 (1988). ArticleCASPubMedGoogle Scholar
- Gan, L. & Jensen, G. J. Electron tomography of cells. Q. Rev. Biophys.45, 27–56 (2012). ArticleCASPubMedGoogle Scholar
- Lucic, V., Rigort, A. & Baumeister, W. Cryo-electron tomography: the challenge of doing structural biology in situ. J. Cell Biol.202, 407–419 (2013). ArticleCASPubMedPubMed CentralGoogle Scholar
- Fridman, K., Mader, A., Zwerger, M., Elia, N. & Medalia, O. Advances in tomography: probing the molecular architecture of cells. Nat. Rev. Mol. Cell Biol.13, 736–742 (2012). ArticleCASPubMedGoogle Scholar
- Jensen, G. J. & Briegel, A. How electron cryotomography is opening a new window onto prokaryotic ultrastructure. Curr. Opin. Struct. Biol.17, 260–267 (2007). ArticleCASPubMedGoogle Scholar
- Zuber, B. et al. Direct visualization of the outer membrane of mycobacteria and corynebacteria in their native state. J. Bacteriol.190, 5672–5680 (2008). ArticleCASPubMedPubMed CentralGoogle Scholar
- Hoffmann, C., Leis, A., Niederweis, M., Plitzko, J. M. & Engelhardt, H. Disclosure of the mycobacterial outer membrane: cryo-electron tomography and vitreous sections reveal the lipid bilayer structure. Proc. Natl Acad. Sci. USA105, 3963–3967 (2008). ArticleCASPubMedPubMed CentralGoogle Scholar
- Gan, L., Chen, S. & Jensen, G. J. Molecular organization of Gram-negative peptidoglycan. Proc. Natl Acad. Sci. USA105, 18953–18957 (2008). ArticleCASPubMedPubMed CentralGoogle Scholar
- Beeby, M., Gumbart, J. C., Roux, B. & Jensen, G. J. Architecture and assembly of the Gram-positive cell wall. Mol. Microbiol.88, 664–672 (2013). ArticleCASPubMedPubMed CentralGoogle Scholar
- Tocheva, E. I. et al. Peptidoglycan transformations during Bacillus subtilis sporulation. Mol. Microbiol.88, 673–686 (2013). ArticleCASPubMedPubMed CentralGoogle Scholar
- Turner, R. D., Vollmer, W. & Foster, S. J. Different walls for rods and balls: the diversity of peptidoglycan. Mol. Microbiol.91, 862–874 (2014). ArticleCASPubMedPubMed CentralGoogle Scholar
- Pilhofer, M. et al. Discovery of chlamydial peptidoglycan reveals bacteria with murein sacculi but without FtsZ. Nat. Commun.4, 2856 (2013). ArticlePubMedCASGoogle Scholar
- Swulius, M. T. et al. Long helical filaments are not seen encircling cells in electron cryotomograms of rod-shaped bacteria. Biochem. Biophys. Res. Commun.407, 650–655 (2011). ArticleCASPubMedPubMed CentralGoogle Scholar
- Swulius, M. T. & Jensen, G. J. The helical MreB cytoskeleton in Escherichia coli MC1000/pLE7 is an artifact of the N-terminal yellow fluorescent protein tag. J. Bacteriol.194, 6382–6386 (2012). ArticleCASPubMedPubMed CentralGoogle Scholar
- Dominguez-Escobar, J. et al. Processive movement of MreB-associated cell wall biosynthetic complexes in bacteria. Science333, 225–228 (2011). ArticleCASPubMedGoogle Scholar
- Garner, E. C. et al. Coupled, circumferential motions of the cell wall synthesis machinery and MreB filaments in B. subtilis. Science333, 222–225 (2011). ArticleCASPubMedPubMed CentralGoogle Scholar
- Briegel, A. et al. Multiple large filament bundles observed in Caulobacter crescentus by electron cryotomography. Mol. Microbiol.62, 5–14 (2006). ArticleCASPubMedGoogle Scholar
- Ingerson-Mahar, M., Briegel, A., Werner, J. N., Jensen, G. J. & Gitai, Z. The metabolic enzyme CTP synthase forms cytoskeletal filaments. Nat. Cell Biol.12, 739–746 (2010). ArticleCASPubMedPubMed CentralGoogle Scholar
- Barry, R. M. & Gitai, Z. Self-assembling enzymes and the origins of the cytoskeleton. Curr. Opin. Microbiol.14, 704–711 (2011). ArticleCASPubMedPubMed CentralGoogle Scholar
- Kuhn, J. et al. Bactofilins, a ubiquitous class of cytoskeletal proteins mediating polar localization of a cell wall synthase in Caulobacter crescentus. EMBO J.29, 327–339 (2010). ArticlePubMedCASGoogle Scholar
- Scheffel, A. et al. An acidic protein aligns magnetosomes along a filamentous structure in magnetotactic bacteria. Nature440, 110–114 (2006). ArticleCASPubMedGoogle Scholar
- Komeili, A., Li, Z., Newman, D. K. & Jensen, G. J. Magnetosomes are cell membrane invaginations organized by the actin-like protein MamK. Science311, 242–245 (2006). Together with Ref. 27, these papers describe the cytoskeletal filaments of MamK that align magnetosomes into chains.ArticleCASPubMedGoogle Scholar
- Scheffel, A. & Schuler, D. The acidic repetitive domain of the Magnetospirillum gryphiswaldense MamJ protein displays hypervariability but is not required for magnetosome chain assembly. J. Bacteriol.189, 6437–6446 (2007). ArticleCASPubMedPubMed CentralGoogle Scholar
- Katzmann, E., Scheffel, A., Gruska, M., Plitzko, J. M. & Schuler, D. Loss of the actin-like protein MamK has pleiotropic effects on magnetosome formation and chain assembly in Magnetospirillum gryphiswaldense. Mol. Microbiol.77, 208–224 (2010). ArticleCASPubMedGoogle Scholar
- Draper, O. et al. MamK, a bacterial actin, forms dynamic filaments in vivo that are regulated by the acidic proteins MamJ and LimJ. Mol. Microbiol.82, 342–354 (2011). ArticleCASPubMedPubMed CentralGoogle Scholar
- Jenkins, C. et al. Genes for the cytoskeletal protein tubulin in the bacterial genus Prosthecobacter. Proc. Natl Acad. Sci. USA99, 17049–17054 (2002). ArticleCASPubMedPubMed CentralGoogle Scholar
- Sontag, C. A., Staley, J. T. & Erickson, H. P. In vitro assembly and GTP hydrolysis by bacterial tubulins BtubA and BtubB. J. Cell Biol.169, 233–238 (2005). ArticleCASPubMedPubMed CentralGoogle Scholar
- Pilhofer, M., Ladinsky, M. S., McDowall, A. W., Petroni, G. & Jensen, G. J. Microtubules in bacteria: Ancient tubulins build a five-protofilament homolog of the eukaryotic cytoskeleton. PLoS Biol.9, e1001213 (2011). This work describes bacterial microtubules containing five protofilaments.ArticleCASPubMedPubMed CentralGoogle Scholar
- Bohm, J. et al. Toward detecting and identifying macromolecules in a cellular context: template matching applied to electron tomograms. Proc. Natl Acad. Sci. USA97, 14245–14250 (2000). ArticleCASPubMedPubMed CentralGoogle Scholar
- Frangakis, A. S. et al. Identification of macromolecular complexes in cryoelectron tomograms of phantom cells. Proc. Natl Acad. Sci. USA99, 14153–14158 (2002). ArticleCASPubMedPubMed CentralGoogle Scholar
- Seybert, A., Herrmann, R. & Frangakis, A. S. Structural analysis of Mycoplasma pneumoniae by cryo-electron tomography. J. Struct. Biol.156, 342–354 (2006). ArticleCASPubMedGoogle Scholar
- Malmstrom, J. et al. Proteome-wide cellular protein concentrations of the human pathogen Leptospira interrogans. Nature460, 762–765 (2009). ArticlePubMedPubMed CentralCASGoogle Scholar
- Ortiz, J. O., Forster, F., Kurner, J., Linaroudis, A. A. & Baumeister, W. Mapping 70S ribosomes in intact cells by cryoelectron tomography and pattern recognition. J. Struct. Biol.156, 334–341 (2006). ArticleCASPubMedGoogle Scholar
- Comolli, L. R., Baker, B. J., Downing, K. H., Siegerist, C. E. & Banfield, J. F. Three-dimensional analysis of the structure and ecology of a novel, ultra-small archaeon. ISME J.3, 159–167 (2009). ArticleCASPubMedGoogle Scholar
- Beck, M. et al. Visual proteomics of the human pathogen Leptospira interrogans. Nat. Methods6, 817–823 (2009). ArticleCASPubMedPubMed CentralGoogle Scholar
- Kuhner, S. et al. Proteome organization in a genome-reduced bacterium. Science326, 1235–1240 (2009). Together with Ref. 41, this study uses visual proteomics to survey thein situlocations of protein complexes in intact cells.ArticlePubMedCASGoogle Scholar
- Martinez-Planells, A. et al. Determination of the topography and biometry of chlorosomes by atomic force microscopy. Photosynth Res.71, 83–90 (2002). ArticleCASPubMedGoogle Scholar
- Psencik, J. et al. Structure of chlorosomes from the green filamentous bacterium Chloroflexus aurantiacus. J. Bacteriol.191, 6701–6708 (2009). ArticleCASPubMedPubMed CentralGoogle Scholar
- Kudryashev, M., Aktoudianaki, A., Dedoglou, D., Stahlberg, H. & Tsiotis, G. The ultrastructure of Chlorobaculum tepidum revealed by cryo-electron tomography. Biochim. Biophys. Acta1837, 1635–1642 (2014). ArticleCASPubMedGoogle Scholar
- Ting, C. S., Hsieh, C., Sundararaman, S., Mannella, C. & Marko, M. Cryo-electron tomography reveals the comparative three-dimensional architecture of Prochlorococcus, a globally important marine cyanobacterium. J. Bacteriol.189, 4485–4493 (2007). ArticleCASPubMedPubMed CentralGoogle Scholar
- Konorty, M., Kahana, N., Linaroudis, A., Minsky, A. & Medalia, O. Structural analysis of photosynthetic membranes by cryo-electron tomography of intact Rhodopseudomonas viridis cells. J. Struct. Biol.161, 393–400 (2008). ArticleCASPubMedGoogle Scholar
- Konorty, M. et al. Photosynthetic system in Blastochloris viridis revisited. Biochemistry48, 4753–4761 (2009). ArticleCASPubMedGoogle Scholar
- Tucker, J. D. et al. Membrane invagination in Rhodobacter sphaeroides is initiated at curved regions of the cytoplasmic membrane, then forms both budded and fully detached spherical vesicles. Mol. Microbiol.76, 833–847 (2010). ArticleCASPubMedGoogle Scholar
- Ellis, R. J. The most abundant protein in the world. Trends Biochem. Sci.4, 241–244 (1979). ArticleCASGoogle Scholar
- Schmid, M. F. et al. Structure of Halothiobacillus neapolitanus carboxysomes by cryo-electron tomography. J. Mol. Biol.364, 526–535 (2006). ArticleCASPubMedPubMed CentralGoogle Scholar
- Iancu, C. V. et al. The structure of isolated Synechococcus strain WH8102 carboxysomes as revealed by electron cryotomography. J. Mol. Biol.372, 764–773 (2007). ArticleCASPubMedPubMed CentralGoogle Scholar
- Iancu, C. V. et al. Organization, structure, and assembly of α-carboxysomes determined by electron cryotomography of intact cells. J. Mol. Biol.396, 105–117 (2010). ArticleCASPubMedGoogle Scholar
- Tocheva, E. I. et al. Structure and expression of propanediol utilization microcompartments in Acetonema longum. J. Bacteriol.196, 1651–1658 (2014). ArticlePubMedPubMed CentralCASGoogle Scholar
- Butan, C. et al. Spiral architecture of the nucleoid in Bdellovibrio bacteriovorus. J. Bacteriol.193, 1341–1350 (2011). This paper describes the organization of the nucleoid in an intact cell.ArticleCASPubMedGoogle Scholar
- Raddi, G. et al. Three-dimensional structures of pathogenic and saprophytic Leptospira species revealed by cryo-electron tomography. J. Bacteriol.194, 1299–1306 (2012). ArticleCASPubMedPubMed CentralGoogle Scholar
- Comolli, L. R. et al. A portable cryo-plunger for on-site intact cryogenic microscopy sample preparation in natural environments. Microsc. Res. Tech.75, 829–836 (2012). ArticlePubMedGoogle Scholar
- Luef, B. et al. Diverse uncultivated ultra-small bacterial cells in groundwater. Nat. Commun.6, 6372 (2015). ArticleCASPubMedGoogle Scholar
- Schlimpert, S. et al. General protein diffusion barriers create compartments within bacterial cells. Cell151, 1270–1282 (2012). ArticleCASPubMedPubMed CentralGoogle Scholar
- Comolli, L. R., Luef, B. & Chan, C. S. High-resolution 2D and 3D cryo-TEM reveals structural adaptations of two stalk-forming bacteria to an Fe-oxidizing lifestyle. Environ. Microbiol.13, 2915–2929 (2011). ArticleCASPubMedGoogle Scholar
- Luef, B. et al. Iron-reducing bacteria accumulate ferric oxyhydroxide nanoparticle aggregates that may support planktonic growth. ISME J.7, 338–350 (2013). ArticleCASPubMedGoogle Scholar
- Shetty, A., Chen, S., Tocheva, E. I., Jensen, G. J. & Hickey, W. J. Nanopods: a new bacterial structure and mechanism for deployment of outer membrane vesicles. PLoS ONE6, e20725 (2011). ArticleCASPubMedPubMed CentralGoogle Scholar
- Murphy, G. E., Leadbetter, J. R. & Jensen, G. J. In situ structure of the complete Treponema primitia flagellar motor. Nature442, 1062–1064 (2006). ArticleCASPubMedGoogle Scholar
- Liu, J. et al. Intact flagellar motor of Borrelia burgdorferi revealed by cryo-electron tomography: evidence for stator ring curvature and rotor/C-ring assembly flexion. J. Bacteriol.191, 5026–5036 (2009). ArticleCASPubMedPubMed CentralGoogle Scholar
- Liu, J. et al. Cellular architecture of Treponema pallidum: novel flagellum, periplasmic cone, and cell envelope as revealed by cryo electron tomography. J. Mol. Biol.403, 546–561 (2010). ArticleCASPubMedPubMed CentralGoogle Scholar
- Chen, S. et al. Structural diversity of bacterial flagellar motors. EMBO J.30, 2972–2981 (2011). This paper describes the structural diversity of flagellar motors in 11 bacterial species.ArticleCASPubMedPubMed CentralGoogle Scholar
- Hosogi, N., Shigematsu, H., Terashima, H., Homma, M. & Nagayama, K. Zernike phase contrast cryo-electron tomography of sodium-driven flagellar hook-basal bodies from Vibrio alginolyticus. J. Struct. Biol.173, 67–76 (2011). ArticleCASPubMedGoogle Scholar
- Zhao, X. et al. Cryoelectron tomography reveals the sequential assembly of bacterial flagella in Borrelia burgdorferi. Proc. Natl Acad. Sci. USA110, 14390–14395 (2013). This work uses high-resolution subtomogram averaging and mutant analysis to construct a detailed structural picture of flagellar assemblyin vivoinB. burgdorferi. ArticleCASPubMedPubMed CentralGoogle Scholar
- Izard, J., Hsieh, C. E., Limberger, R. J., Mannella, C. A. & Marko, M. Native cellular architecture of Treponema denticola revealed by cryo-electron tomography. J. Struct. Biol.163, 10–17 (2008). ArticleCASPubMedPubMed CentralGoogle Scholar
- Kudryashev, M. et al. Comparative cryo-electron tomography of pathogenic Lyme disease spirochetes. Mol. Microbiol.71, 1415–1434 (2009). ArticleCASPubMedGoogle Scholar
- Xu, H., Raddi, G., Liu, J., Charon, N. W. & Li, C. Chemoreceptors and flagellar motors are subterminally located in close proximity at the two cell poles in spirochetes. J. Bacteriol.193, 2652–2656 (2011). ArticleCASPubMedPubMed CentralGoogle Scholar
- Motaleb, M. A. et al. Borrelia burgdorferi periplasmic flagella have both skeletal and motility functions. Proc. Natl Acad. Sci. USA97, 10899–10904 (2000). ArticleCASPubMedPubMed CentralGoogle Scholar
- Charon, N. W. et al. The flat-ribbon configuration of the periplasmic flagella of Borrelia burgdorferi and its relationship to motility and morphology. J. Bacteriol.191, 600–607 (2009). ArticleCASPubMedGoogle Scholar
- Sze, C. W. et al. Carbon storage regulator A (CsrA(Bb)) is a repressor of Borrelia burgdorferi flagellin protein FlaB. Mol. Microbiol.82, 851–864 (2011). ArticleCASPubMedPubMed CentralGoogle Scholar
- Zhang, K., Tong, B. A., Liu, J. & Li, C. A single-domain FlgJ contributes to flagellar hook and filament formation in the Lyme disease spirochete Borrelia burgdorferi. J. Bacteriol.194, 866–874 (2012). ArticleCASPubMedPubMed CentralGoogle Scholar
- Motaleb, M. A., Pitzer, J. E., Sultan, S. Z. & Liu, J. A novel gene inactivation system reveals altered periplasmic flagellar orientation in a Borrelia burgdorferi fliL mutant. J. Bacteriol.193, 3324–3331 (2011). ArticleCASPubMedPubMed CentralGoogle Scholar
- Sultan, S. Z. et al. Motor rotation is essential for the formation of the periplasmic flagellar ribbon, cellular morphology, and Borrelia burgdorferi persistence within Ixodes scapularis tick and murine hosts. Infect. Immun.83, 1765–1777 (2015). ArticleCASPubMedPubMed CentralGoogle Scholar
- Murphy, G. E., Matson, E. G., Leadbetter, J. R., Berg, H. C. & Jensen, G. J. Novel ultrastructures of Treponema primitia and their implications for motility. Mol. Microbiol.67, 1184–1195 (2008). ArticleCASPubMedPubMed CentralGoogle Scholar
- Ruan, J. et al. Architecture of a flagellar apparatus in the fast-swimming magnetotactic bacterium MO-1. Proc. Natl Acad. Sci. USA109, 20643–20648 (2012). ArticleCASPubMedPubMed CentralGoogle Scholar
- Liu, J., McBride, M. J. & Subramaniam, S. Cell surface filaments of the gliding bacterium Flavobacterium johnsoniae revealed by cryo-electron tomography. J. Bacteriol.189, 7503–7506 (2007). ArticleCASPubMedPubMed CentralGoogle Scholar
- Kurner, J., Frangakis, A. S. & Baumeister, W. Cryo-electron tomography reveals the cytoskeletal structure of Spiroplasma melliferum. Science307, 436–438 (2005). This early description of bacterial cytoskeletal filaments proposed a model for motility of helical Mollicutes based on cytoskeletal ribbons.ArticlePubMedCASGoogle Scholar
- Henderson, G. P. & Jensen, G. J. Three-dimensional structure of Mycoplasma pneumoniae's attachment organelle and a model for its role in gliding motility. Mol. Microbiol.60, 376–385 (2006). ArticleCASPubMedGoogle Scholar
- Jasnin, M. et al. Three-dimensional architecture of actin filaments in Listeria monocytogenes comet tails. Proc. Natl Acad. Sci. USA110, 20521–20526 (2013). ArticleCASPubMedPubMed CentralGoogle Scholar
- Byrne, M. E. et al. Desulfovibrio magneticus RS-1 contains an iron- and phosphorus-rich organelle distinct from its bullet-shaped magnetosomes. Proc. Natl Acad. Sci. USA107, 12263–12268 (2010). ArticleCASPubMedPubMed CentralGoogle Scholar
- Abreu, F. et al. Cryo-electron tomography of the magnetotactic vibrio Magnetovibrio blakemorei: insights into the biomineralization of prismatic magnetosomes. J. Struct. Biol.181, 162–168 (2013). ArticleCASPubMedGoogle Scholar
- Zhang, P., Khursigara, C. M., Hartnell, L. M. & Subramaniam, S. Direct visualization of Escherichia coli chemotaxis receptor arrays using cryo-electron microscopy. Proc. Natl Acad. Sci. USA104, 3777–3781 (2007). ArticleCASPubMedPubMed CentralGoogle Scholar
- Khursigara, C. M., Wu, X., Zhang, P., Lefman, J. & Subramaniam, S. Role of HAMP domains in chemotaxis signaling by bacterial chemoreceptors. Proc. Natl Acad. Sci. USA105, 16555–16560 (2008). ArticleCASPubMedPubMed CentralGoogle Scholar
- Briegel, A. et al. Location and architecture of the Caulobacter crescentus chemoreceptor array. Mol. Microbiol.69, 30–41 (2008). ArticleCASPubMedPubMed CentralGoogle Scholar
- Khursigara, C. M., Wu, X. & Subramaniam, S. Chemoreceptors in Caulobacter crescentus: trimers of receptor dimers in a partially ordered hexagonally packed array. J. Bacteriol.190, 6805–6810 (2008). ArticleCASPubMedPubMed CentralGoogle Scholar
- Briegel, A., Beeby, M., Thanbichler, M. & Jensen, G. J. Activated chemoreceptor arrays remain intact and hexagonally packed. Mol. Microbiol.82, 748–757 (2011). ArticleCASPubMedPubMed CentralGoogle Scholar
- Khursigara, C. M. et al. Lateral density of receptor arrays in the membrane plane influences sensitivity of the E. coli chemotaxis response. EMBO J.30, 1719–1729 (2011). ArticleCASPubMedPubMed CentralGoogle Scholar
- Zhang, K. et al. Two CheW coupling proteins are essential in a chemosensory pathway of Borrelia burgdorferi. Mol. Microbiol.85, 782–794 (2012). ArticleCASPubMedPubMed CentralGoogle Scholar
- Yamaichi, Y. et al. A multidomain hub anchors the chromosome segregation and chemotactic machinery to the bacterial pole. Genes Dev.26, 2348–2360 (2012). ArticleCASPubMedPubMed CentralGoogle Scholar
- Briegel, A. et al. Universal architecture of bacterial chemoreceptor arrays. Proc. Natl Acad. Sci. USA106, 17181–17186 (2009). ArticleCASPubMedPubMed CentralGoogle Scholar
- Briegel, A. et al. Structural conservation of chemotaxis machinery across Archaea and Bacteria. Environ. Microbiol. Rep.7, 414–419 (2015). ArticleCASPubMedPubMed CentralGoogle Scholar
- Briegel, A. et al. Structure of bacterial cytoplasmic chemoreceptor arrays and implications for chemotactic signaling. eLife3, e02151 (2014). ArticlePubMedPubMed CentralCASGoogle Scholar
- Briegel, A. et al. Bacterial chemoreceptor arrays are hexagonally packed trimers of receptor dimers networked by rings of kinase and coupling proteins. Proc. Natl Acad. Sci. USA109, 3766–3771 (2012). ArticleCASPubMedPubMed CentralGoogle Scholar
- Liu, J. et al. Molecular architecture of chemoreceptor arrays revealed by cryoelectron tomography of Escherichia coli minicells. Proc. Natl Acad. Sci. USA109, E1481–E1488 (2012). Together with Ref. 97, this study provides a detailed structural model of the bacterial chemoreceptor array.CASPubMedPubMed CentralGoogle Scholar
- Briegel, A. et al. The mobility of two kinase domains in the Escherichia coli chemoreceptor array varies with signalling state. Mol. Microbiol.89, 831–841 (2013). ArticleCASPubMedPubMed CentralGoogle Scholar
- Briegel, A. et al. New insights into bacterial chemoreceptor array structure and assembly from electron cryotomography. Biochemistry53, 1575–1585 (2014). ArticleCASPubMedGoogle Scholar
- Ebersbach, G., Briegel, A. & Jensen, G. J. & Jacobs-Wagner, C. A self-associating protein critical for chromosome attachment, division, and polar organization in Caulobacter. Cell134, 956–968 (2008). ArticleCASPubMedPubMed CentralGoogle Scholar
- Bowman, G. R. et al. Caulobacter PopZ forms a polar subdomain dictating sequential changes in pole composition and function. Mol. Microbiol.76, 173–189 (2010). ArticleCASPubMedPubMed CentralGoogle Scholar
- Salje, J., Zuber, B. & Lowe, J. Electron cryomicroscopy of E. coli reveals filament bundles involved in plasmid DNA segregation. Science323, 509–512 (2009). ArticleCASPubMedGoogle Scholar
- Bharat, T. A., Murshudov, G. N., Sachse, C. & Lowe, J. Structures of actin-like ParM filaments show architecture of plasmid-segregating spindles. Nature523, 106–110 (2015). This study reveals plasmid segregation by antiparallel doublets of ParM filaments.ArticleCASPubMedPubMed CentralGoogle Scholar
- Aylett, C. H., Wang, Q., Michie, K. A., Amos, L. A. & Lowe, J. Filament structure of bacterial tubulin homologue TubZ. Proc. Natl Acad. Sci. USA107, 19766–19771 (2010). ArticleCASPubMedPubMed CentralGoogle Scholar
- Szwedziak, P., Wang, Q., Freund, S. M. & Lowe, J. FtsA forms actin-like protofilaments. EMBO J.31, 2249–2260 (2012). ArticleCASPubMedPubMed CentralGoogle Scholar
- Judd, E. M. et al. Distinct constrictive processes, separated in time and space, divide Caulobacter inner and outer membranes. J. Bacteriol.187, 6874–6882 (2005). ArticleCASPubMedPubMed CentralGoogle Scholar
- Li, Z., Trimble, M. J., Brun, Y. V. & Jensen, G. J. The structure of FtsZ filaments in vivo suggests a force-generating role in cell division. EMBO J.26, 4694–4708 (2007). ArticleCASPubMedPubMed CentralGoogle Scholar
- Lu, C., Reedy, M. & Erickson, H. P. Straight and curved conformations of FtsZ are regulated by GTP hydrolysis. J. Bacteriol.182, 164–170 (2000). ArticleCASPubMedPubMed CentralGoogle Scholar
- Szwedziak, P., Wang, Q., Bharat, T. A., Tsim, M. & Lowe, J. Architecture of the ring formed by the tubulin homologue FtsZ in bacterial cell division. eLife3, e04601 (2014). ArticlePubMedPubMed CentralGoogle Scholar
- Ghosal, D., Trambaiolo, D., Amos, L. A. & Lowe, J. MinCD cell division proteins form alternating copolymeric cytomotive filaments. Nat. Commun.5, 5341 (2014). ArticleCASPubMedGoogle Scholar
- Moll, A., Schlimpert, S., Briegel, A. & Jensen, G. J. & Thanbichler, M. DipM, a new factor required for peptidoglycan remodelling during cell division in Caulobacter crescentus. Mol. Microbiol.77, 90–107 (2010). ArticlePubMedPubMed CentralCASGoogle Scholar
- Goley, E. D., Comolli, L. R., Fero, K. E., Downing, K. H. & Shapiro, L. DipM links peptidoglycan remodelling to outer membrane organization in Caulobacter. Mol. Microbiol.77, 56–73 (2010). ArticleCASPubMedPubMed CentralGoogle Scholar
- Dobro, M. J. et al. Electron cryotomography of ESCRT assemblies and dividing Sulfolobus cells suggests that spiraling filaments are involved in membrane scission. Mol. Biol. Cell24, 2319–2327 (2013). ArticleCASPubMedPubMed CentralGoogle Scholar
- Katzmann, E. et al. Magnetosome chains are recruited to cellular division sites and split by asymmetric septation. Mol. Microbiol.82, 1316–1329 (2011). ArticlePubMedGoogle Scholar
- Comolli, L. R., Kundmann, M. & Downing, K. H. Characterization of intact subcellular bodies in whole bacteria by cryo-electron tomography and spectroscopic imaging. J. Microsc.223, 40–52 (2006). ArticleCASPubMedGoogle Scholar
- Tocheva, E. I. et al. Polyphosphate storage during sporulation in the Gram-negative bacterium Acetonema longum. J. Bacteriol.195, 3940–3946 (2013). ArticleCASPubMedPubMed CentralGoogle Scholar
- Beeby, M., Cho, M., Stubbe, J. & Jensen, G. J. Growth and localization of polyhydroxybutyrate granules in Ralstonia eutropha. J. Bacteriol.194, 1092–1099 (2012). ArticleCASPubMedPubMed CentralGoogle Scholar
- Toso, D. B., Henstra, A. M., Gunsalus, R. P. & Zhou, Z. H. Structural mass and elemental analyses of storage granules in methanogenic archaeal cells. Environ. Microbiol.13, 2587–2599 (2011). ArticleCASPubMedPubMed CentralGoogle Scholar
- Tocheva, E. I. et al. Peptidoglycan remodeling and conversion of an inner membrane into an outer membrane during sporulation. Cell146, 799–812 (2011). This work reveals the interconversion of inner and outer membranes during Gram-negative sporulation, suggesting a potential evolutionary source of the outer membrane.ArticleCASPubMedPubMed CentralGoogle Scholar
- Schrempf, H., Koebsch, I., Walter, S., Engelhardt, H. & Meschke, H. Extracellular Streptomyces vesicles: amphorae for survival and defence. Microb. Biotechnol.4, 286–299 (2011). ArticleCASPubMedPubMed CentralGoogle Scholar
- Sleytr, U. B., Messner, P., Pum, D. & Sara, M. Crystalline bacterial cell surface layers. Mol. Microbiol.10, 911–916 (1993). ArticleCASPubMedGoogle Scholar
- Grimm, R. et al. Electron tomography of ice-embedded prokaryotic cells. Biophys. J.74, 1031–1042 (1998). ArticleCASPubMedPubMed CentralGoogle Scholar
- Amat, F. et al. Analysis of the intact surface layer of Caulobacter crescentus by cryo-electron tomography. J. Bacteriol.192, 5855–5865 (2010). ArticleCASPubMedPubMed CentralGoogle Scholar
- Nickell, S. Hegerl, R., Baumeister, W. & Rachel, R. Pyrodictium cannulae enter the periplasmic space but do not enter the cytoplasm, as revealed by cryo-electron tomography. J. Struct. Biol.141, 34–42 (2003). ArticlePubMedGoogle Scholar
- Junglas, B. et al. Ignicoccus hospitalis and Nanoarchaeum equitans: ultrastructure, cell-cell interaction, and 3D reconstruction from serial sections of freeze-substituted cells and by electron cryotomography. Arch. Microbiol.190, 395–408 (2008). ArticleCASPubMedPubMed CentralGoogle Scholar
- Comolli, L. R. & Banfield, J. F. Inter-species interconnections in acid mine drainage microbial communities. Front. Microbiol.5, 367 (2014). PubMedPubMed CentralGoogle Scholar
- Moissl, C. Rachel, R., Briegel, A., Engelhardt, H. & Huber, R. The unique structure of archaeal 'hami', highly complex cell appendages with nano-grappling hooks. Mol. Microbiol.56, 361–370 (2005). ArticleCASPubMedGoogle Scholar
- Basler, M., Pilhofer, M., Henderson, G. P., Jensen, G. J. & Mekalanos, J. J. Type VI secretion requires a dynamic contractile phage tail-like structure. Nature483, 182–186 (2012). This study reveals the phage tail-like contractile mechanism of the T6SS.ArticleCASPubMedPubMed CentralGoogle Scholar
- Chang, Y. W. et al. Correlated cryogenic photoactivated localization microscopy and cryo-electron tomography. Nat. Methods11, 737–739 (2014). This paper describes the technique of correlated cryo-PALM and ECT.ArticleCASPubMedPubMed CentralGoogle Scholar
- Shikuma, N. J. et al. Marine tubeworm metamorphosis induced by arrays of bacterial phage tail-like structures. Science343, 529–533 (2014). ArticleCASPubMedPubMed CentralGoogle Scholar
- Borgnia, M. J., Subramaniam, S. & Milne, J. L. Three-dimensional imaging of the highly bent architecture of Bdellovibrio bacteriovorus by using cryo-electron tomography. J. Bacteriol.190, 2588–2596 (2008). ArticleCASPubMedPubMed CentralGoogle Scholar
- Muller, A. et al. Ultrastructure and complex polar architecture of the human pathogen Campylobacter jejuni. Microbiologyopen3, 702–710 (2014). ArticlePubMedPubMed CentralCASGoogle Scholar
- Izard, J. et al. Cryo-electron tomography elucidates the molecular architecture of Treponema pallidum, the syphilis spirochete. J. Bacteriol.191, 7566–7580 (2009). ArticleCASPubMedPubMed CentralGoogle Scholar
- Kudryashev, M. et al. Evidence of direct cell-cell fusion in Borrelia by cryogenic electron tomography. Cell. Microbiol.13, 731–741 (2011). ArticleCASPubMedGoogle Scholar
- Abrusci, P. et al. Architecture of the major component of the type III secretion system export apparatus. Nat. Struct. Mol. Biol.20, 99–104 (2013). ArticleCASPubMedGoogle Scholar
- Kawamoto, A. et al. Common and distinct structural features of Salmonella injectisome and flagellar basal body. Sci. Rep.3, 3369 (2013). ArticlePubMedPubMed CentralGoogle Scholar
- Kudryashev, M. et al. In situ structural analysis of the Yersinia enterocolitica injectisome. eLife2, e00792 (2013). ArticlePubMedPubMed CentralCASGoogle Scholar
- Kudryashev, M. et al. Yersinia enterocolitica type III secretion injectisomes form regularly spaced clusters, which incorporate new machines upon activation. Mol. Microbiol.95, 875–884 (2014). ArticleCASGoogle Scholar
- Nans, A., Saibil, H. R. & Hayward, R. D. Pathogen–host reorganization during Chlamydia invasion revealed by cryo-electron tomography. Cell. Microbiol.16, 1457–1472 (2014). ArticleCASPubMedPubMed CentralGoogle Scholar
- Hu, B. et al. Visualization of the type III secretion sorting platform of Shigella flexneri. Proc. Natl Acad. Sci. USA112, 1047–1052 (2015). This work provides a pseudo-atomic model of the pathogenic T3SS, including the substrate sorting platform.ArticleCASPubMedPubMed CentralGoogle Scholar
- Huang, Z. et al. Cryo-electron tomography of Chlamydia trachomatis gives a clue to the mechanism of outer membrane changes. J. Electron. Microsc. (Tokyo)59, 237–241 (2010). ArticleGoogle Scholar
- Wilkat, M., Herdoiza, E., Forsbach-Birk, V., Walther, P. & Essig, A. Electron tomography and cryo-SEM characterization reveals novel ultrastructural features of host-parasite interaction during Chlamydia abortus infection. Histochem. Cell Biol.142, 171–184 (2014). ArticleCASPubMedGoogle Scholar
- LaRocca, T. J. et al. The bactericidal effect of a complement-independent antibody is osmolytic and specific to Borrelia. Proc. Natl Acad. Sci. USA106, 10752–10757 (2009). ArticleCASPubMedPubMed CentralGoogle Scholar
- Watkins, B. Y. et al. Mycobacterium marinum SecA2 promotes stable granulomas and induces tumor necrosis factor-α in vivo. Infect. Immun.80, 3512–3520 (2012). ArticleCASPubMedPubMed CentralGoogle Scholar
- Koning, R. I. et al. Cryo-electron tomography analysis of membrane vesicles from Acinetobacter baumannii ATCC19606 T. Res. Microbiol.164, 397–405 (2013). ArticleCASPubMedGoogle Scholar
- Trepout, S. et al. Structure of reconstituted bacterial membrane efflux pump by cryo-electron tomography. Biochim. Biophys. Acta1798, 1953–1960 (2010). ArticleCASPubMedGoogle Scholar
- Wysocki, L. M. & Lavis, L. D. Advances in the chemistry of small molecule fluorescent probes. Curr. Opin. Chem. Biol.15, 752–759 (2011). ArticleCASPubMedGoogle Scholar
- Zhang, W. H., Otting, G. & Jackson, C. J. Protein engineering with unnatural amino acids. Curr. Opin. Struct. Biol.23, 581–587 (2013). ArticleCASPubMedGoogle Scholar
- Mercogliano, C. P. & DeRosier, D. J. Concatenated metallothionein as a clonable gold label for electron microscopy. J. Struct. Biol.160, 70–82 (2007). ArticleCASPubMedPubMed CentralGoogle Scholar
- Wang, Q., Mercogliano, C. P. & Lowe, J. A ferritin-based label for cellular electron cryotomography. Structure19, 147–154 (2011). ArticleCASPubMedGoogle Scholar
- Diestra, E., Fontana, J., Guichard, P., Marco, S. & Risco, C. Visualization of proteins in intact cells with a clonable tag for electron microscopy. J. Struct. Biol.165, 157–168 (2009). ArticleCASPubMedGoogle Scholar
- Bouchet-Marquis, C., Pagratis, M., Kirmse, R. & Hoenger, A. Metallothionein as a clonable high-density marker for cryo-electron microscopy. J. Struct. Biol.177, 119–127 (2012). ArticleCASPubMedGoogle Scholar
- Delgado, L. Martinez, G., Lopez-Iglesias, C. & Mercade, E. Cryo-electron tomography of plunge-frozen whole bacteria and vitreous sections to analyze the recently described bacterial cytoplasmic structure, the Stack. J. Struct. Biol.189, 220–229 (2015). ArticleCASPubMedGoogle Scholar
- Bernadac, A. et al. Structural properties of the tubular appendage spinae from marine bacterium Roseobacter sp. strain YSCB. Sci. Rep.2, 950 (2012). ArticleCASPubMedPubMed CentralGoogle Scholar
- Tivol, W. F., Briegel, A. & Jensen, G. J. An improved cryogen for plunge freezing. Microsc. Microanal.14, 375–379 (2008). ArticleCASPubMedPubMed CentralGoogle Scholar
- Al-Amoudi, A., Norlen, L. P. & Dubochet, J. Cryo-electron microscopy of vitreous sections of native biological cells and tissues. J. Struct. Biol.148, 131–135 (2004). This paper describes the technique of vitreous cryosectioning to expand the power of ECT to thicker biological samples.ArticleCASPubMedGoogle Scholar
- Al-Amoudi, A., Studer, D. & Dubochet, J. Cutting artefacts and cutting process in vitreous sections for cryo-electron microscopy. J. Struct. Biol.150, 109–121 (2005). ArticleCASPubMedGoogle Scholar
- Dubochet, J. et al. How to “read” a vitreous section. Methods Cell Biol.79, 385–406 (2007). ArticleCASPubMedGoogle Scholar
- Marko, M., Hsieh, C., Schalek, R., Frank, J. & Mannella, C. Focused-ion-beam thinning of frozen-hydrated biological specimens for cryo-electron microscopy. Nat. Methods4, 215–217 (2007). ArticleCASPubMedGoogle Scholar
- Rigort, A. et al. Focused ion beam micromachining of eukaryotic cells for cryoelectron tomography. Proc. Natl Acad. Sci. USA109, 4449–4454 (2012). This paper illustrates the power of FIB milling to enable high-resolution ECT of thick cells without the artefacts introduced by vitreous cryosectioning.ArticleCASPubMedPubMed CentralGoogle Scholar
- Rigort, A., Villa, E., Bauerlein, F. J., Engel, B. D. & Plitzko, J. M. Integrative approaches for cellular cryo-electron tomography: correlative imaging and focused ion beam micromachining. Methods Cell Biol.111, 259–281 (2012). ArticleCASPubMedGoogle Scholar
- Strunk, K. M., Wang, K., Ke, D., Gray, J. L. & Zhang, P. Thinning of large mammalian cells for cryo-TEM characterization by cryo-FIB milling. J. Microsc.247, 220–227 (2012). ArticleCASPubMedPubMed CentralGoogle Scholar
- McMullan, G., Clark, A. T., Turchetta, R. & Faruqi, A. R. Enhanced imaging in low dose electron microscopy using electron counting. Ultramicroscopy109, 1411–1416 (2009). ArticleCASPubMedPubMed CentralGoogle Scholar
- Murata, K. et al. Zernike phase contrast cryo-electron microscopy and tomography for structure determination at nanometer and subnanometer resolutions. Structure18, 903–912 (2010). ArticleCASPubMedPubMed CentralGoogle Scholar
- Dai, W. et al. Zernike phase-contrast electron cryotomography applied to marine cyanobacteria infected with cyanophages. Nat. Protoc.9, 2630–2642 (2014). This study uses Zernike phase contrast ECT to describe the cyanophage maturation process inside cyanobacterial cells.ArticleCASPubMedPubMed CentralGoogle Scholar
- Fukuda, Y., Laugks, U., Lucic, V., Baumeister, W. & Danev, R. Electron cryotomography of vitrified cells with a Volta phase plate. J. Struct. Biol.190, 143–154 (2015). ArticlePubMedGoogle Scholar
- Danev, R., Buijsse, B., Khoshouei, M., Plitzko, J. M. & Baumeister, W. Volta potential phase plate for in-focus phase contrast transmission electron microscopy. Proc. Natl Acad. Sci. USA111, 15635–15640 (2014). This paper describes the Volta phase plate for improving contrast in transmission electron microscopy imaging.ArticleCASPubMedPubMed CentralGoogle Scholar
- Asano, S. et al. Proteasomes. A molecular census of 26S proteasomes in intact neurons. Science347, 439–442 (2015). This paper illustrates the power of Volta phase plate ECT, distinguishing conformational states of a protein complex in an intact eukaryotic cell.ArticleCASPubMedGoogle Scholar
- Hu, G. B., Wei, H., Rice, W. J., Stokes, D. L. & Gottlieb, P. Electron cryo-tomographic structure of cystovirus Φ12. Virology372, 1–9 (2008). ArticleCASPubMedGoogle Scholar
- Leo-Macias, A. et al. Toroidal surface complexes of bacteriophage Φ12 are responsible for host-cell attachment. Virology414, 103–109 (2011). ArticleCASPubMedGoogle Scholar
- Chang, J. T. et al. Visualizing the structural changes of bacteriophage ɛ15 and its Salmonella host during infection. J. Mol. Biol.402, 731–740 (2010). ArticleCASPubMedPubMed CentralGoogle Scholar
- Dai, W. et al. Three-dimensional structure of tropism-switching Bordetella bacteriophage. Proc. Natl Acad. Sci. USA107, 4347–4352 (2010). ArticleCASPubMedPubMed CentralGoogle Scholar
- Hu, B., Margolin, W., Molineux, I. J. & Liu, J. The bacteriophage T7 virion undergoes extensive structural remodeling during infection. Science339, 576–579 (2013). ArticleCASPubMedGoogle Scholar
- Parent, K. N. et al. OmpA and OmpC are critical host factors for bacteriophage Sf6 entry in Shigella. Mol. Microbiol.92, 47–60 (2014). ArticleCASPubMedPubMed CentralGoogle Scholar
- Guerrero-Ferreira, R. C. et al. Alternative mechanism for bacteriophage adsorption to the motile bacterium Caulobacter crescentus. Proc. Natl Acad. Sci. USA108, 9963–9968 (2011). ArticleCASPubMedPubMed CentralGoogle Scholar
- Dent, K. C. et al. The asymmetric structure of an icosahedral virus bound to its receptor suggests a mechanism for genome release. Structure21, 1225–1234 (2013). ArticleCASPubMedPubMed CentralGoogle Scholar
- Bohm, J. et al. FhuA-mediated phage genome transfer into liposomes: a cryo-electron tomography study. Curr. Biol.11, 1168–1175 (2001). This early application of ECT reveals the contractile injection mechanism of the phage tail.ArticleCASPubMedGoogle Scholar
- Liu, X. et al. Structural changes in a marine podovirus associated with release of its genome into Prochlorococcus. Nat. Struct. Mol. Biol.17, 830–836 (2010). ArticleCASPubMedPubMed CentralGoogle Scholar
- Fu, X., Walter, M. H., Paredes, A., Morais, M. C. & Liu, J. The mechanism of DNA ejection in the Bacillus anthracis spore-binding phage 8a revealed by cryo-electron tomography. Virology421, 141–148 (2011). ArticleCASPubMedGoogle Scholar
- Liu, J., Chen, C. Y., Shiomi, D., Niki, H. & Margolin, W. Visualization of bacteriophage P1 infection by cryo-electron tomography of tiny Escherichia coli. Virology417, 304–311 (2011). ArticleCASPubMedGoogle Scholar
- Nemecek, D., Heymann, J. B., Qiao, J., Mindich, L. & Steven, A. C. Cryo-electron tomography of bacteriophage Φ6 procapsids shows random occupancy of the binding sites for RNA polymerase and packaging NTPase. J. Struct. Biol.171, 389–396 (2010). ArticleCASPubMedPubMed CentralGoogle Scholar
- Dai, W. et al. Visualizing virus assembly intermediates inside marine cyanobacteria. Nature502, 707–710 (2013). ArticleCASPubMedPubMed CentralGoogle Scholar
- Nemecek, D. et al. Stepwise expansion of the bacteriophage Φ6 procapsid: possible packaging intermediates. J. Mol. Biol.414, 260–271 (2011). ArticleCASPubMedPubMed CentralGoogle Scholar
- Dewey, J. S. et al. Micron-scale holes terminate the phage infection cycle. Proc. Natl Acad. Sci. USA107, 2219–2223 (2010). ArticleCASPubMedPubMed CentralGoogle Scholar
- Quemin, E. R. et al. First insights into the entry process of hyperthermophilic archaeal viruses. J. Virol.87, 13379–13385 (2013). ArticleCASPubMedPubMed CentralGoogle Scholar
- Fu, C. Y. et al. In vivo assembly of an archaeal virus studied with whole-cell electron cryotomography. Structure18, 1579–1586 (2010). ArticleCASPubMedPubMed CentralGoogle Scholar
- Daum, B. et al. Self-assembly of the general membrane-remodeling protein PVAP into sevenfold virus-associated pyramids. Proc. Natl Acad. Sci. USA111, 3829–3834 (2014). This study reveals the lytic mechanism of pyramidal archaeal viruses.ArticleCASPubMedPubMed CentralGoogle Scholar
- Hong, C. et al. Lemon-shaped halo archaeal virus His1 with uniform tail but variable capsid structure. Proc. Natl Acad. Sci. USA112, 2449–2454 (2015). ArticleCASPubMedPubMed CentralGoogle Scholar
Acknowledgements
The authors apologize that they could not discuss all of the work in this burgeoning field. The authors thank members of the Jensen laboratory for helpful comments on the manuscript, and J. Ding and Y.-W. Chang for producing the accompanying movie. The authors also thank L. Sockett (University of Nottingham) for the gift of the Bdellovibrio bacteriovorus strain imaged in figure 1 and shown in the accompanying movie. Microbial electron cryotomography (ECT) in the Jensen laboatory is supported, in part, by the Howard Hughes Medical Institute, the US National Institutes of Health (grants RO1 GM101425 and RO1 GM094800), the Beckman Institute at Caltech, Caltech's Center for Environmental Microbial Interactions, and gifts to Caltech from the Gordon and Betty Moore Foundation and the Agouron Institute.
Author information
Authors and Affiliations
- Howard Hughes Medical Institute; Division of Biology and Biological Engineering, California Institute of Technology, Catherine M. Oikonomou, Yi-Wei Chang & Grant J. Jensen
- Division of Biology and Biological Engineering, California Institute of Technology, 1200 E. California Blvd., Pasadena, 91125, California, USA Catherine M. Oikonomou, Yi-Wei Chang & Grant J. Jensen
- Catherine M. Oikonomou